1. Morphology
2. Fusion and fission
3. Protein import
4. Functions
- ATP
- Metabolism
- Stress
- Apoptosis
- Others
5. Renewing
Mitochondria are almost ubiquitous organelles in eukaryotic cells. One exception are archeozoa, which do not show mitochondria, probably because they were lost during evolution. Mitochondria were recognized as a fundamental part of eukaryote cells at the end of the 19th century. Altman (1890) described these organelles after fuchsine staining and observation of many eukaryote cell types. He named them bioblasts. In 1914, it was already known that mitochondria can adopt many shapes, such as small sticks, threads, and nets. The electron microscopy showed that mitochondria were made up of a double membrane. In 1962, it was proposed that mitochondria may grow in size and can divide, so the morphology was dynamic. We know now that mitochondria can split, fuse, and form complex and dynamic networks. There are fluorescent markers that allow us to study the mitochondrial dynamics in vivo.
Mitochondria are descendant organelles from ancestral respiratory alpha-proteobacteria that were included in archaean cells (both cell types were prokaryotes) to form the current eukaryote lineage. Thus, it is proposed that mitochondria emerged about 2000 million years ago through endosymbiosis. Ever since, mitochondria have acquired major cell functions: they are the main energy production source, participate in many metabolic pathways, both anabolic and catabolic, in cellular stress responses, are central hubs to regulate and trigger apoptosis (regulated cell death), and influence cell differentiation and survival. During this evolutionary time, many mitochondrial genes were transferred to the nucleus and became part of the nuclear genome.
1. Morphology
The shape of mitochondria is dynamic and may change from long and branched to small ellipsoids. It could be said that there are mitochondria as individual organelles and as a dynamic mitochondrial networks. As a network or isolated organelle, mitochondria are made up of an outer membrane, an inner membrane, an intermembrane space and an inner space known as the mitochondrial matrix, which is enclosed by the inner membrane (Figures 1 and 2).
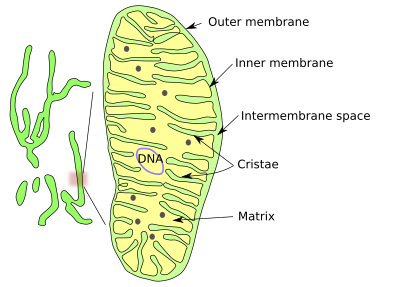
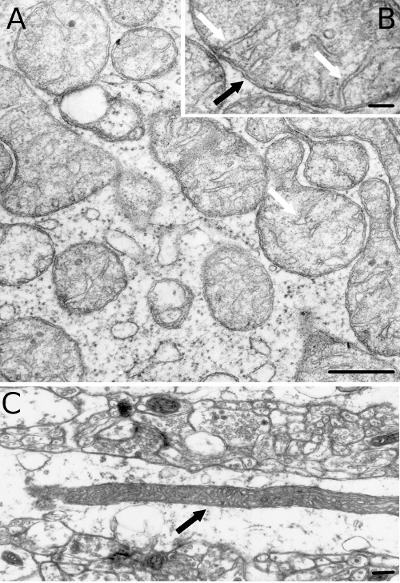
The outer mitochondrial membrane is highly permeable and contains many copies of the protein aquaporin, which is an aqueous channel. This membrane is a kind of sieve, allowing the pass of all those molecules smaller than 5000 Dalton, including small proteins.
On the other hand, the inner mitochondrial membrane is highly impermeable to ions and small molecules. Mitochondria have to make the inner membrane impermeable enough to form a stable proton gradient. The impermeability can be increased by raising the cholesterol concentration in the membrane, but this lowers the fluidity, and mitochondria contain very little cholesterol. The inner mitochondrial membrane needs both hydrophobicity and fluidity to properly develop its function, and for that, mitochondria synthesize cardiolipin, a phospholipid with highly unsaturated fatty acid chains that is translocated to the inner membrane, providing both hydrophobicity and fluidity. In this way, the mitochondrial matrix content is quite different from the cytosol because these inner molecules have to selectively cross both mitochondrial membranes.
Phosphatidylethanolamine and phosphatidylcholine are abundant in mitochondrial membranes, as are cardiolipin. These three membrane lipids are synthesized in mitochodria, but other membrane lipids need to be imported, mostly from the endoplasmic reticulum. It is interesting that part of the phosphatidylethanolamine found in the endoplasmic reticulum comes from mitochondria. The lipid transfer between mitochondria and the endoplasmic reticulum is done at the membrane contact sites by lipid transporters. Cardiolipin and phosphatidylethanolamine account for 40 % of the mitochondrial membrane lipids.
The Inner membrane is heavily folded inward. Each fold is called a mitochondrial crista (cristae in plural). The number and form of cristae is the result of mitochondrial activity, and they are very dynamic depending on the cell type and cell physiology. There are three morphological types of cristae: discoidal, tubular and flattened. Cristae form a distinct compartment from the rest of the inner membrane because they contain a particular set of proteins. The formation, organization and remodeling of cristae ultimately depend on the folding state of the inner membrane and the amount and type of molecules it contains: ATPase dimers, dynamin related proteins, lipid composition, and Mic proteins that allow interactions between the inner and outer membrane. In addition. Cardiolipin shows a wedge-like spatial organization, and this lipid is concentrated in the curved regions of cristae, so it may help to form and modify the mitochodrial inner membrane.
Cristae are connected to one another by mitochondrial inner membrane regions near a parallel to the outer mitochondrial membrane, known as limiting regions. These regions are platforms for lipid exchange and protein importing, bear proteins for mitochondrial fission and fusion, and are places for the assembling of the respiratory chain complexes. Functional respiratory protein complexes and ATP synthases are found in the crista membrane, as are the proteins for the formation of sulfur-iron groups. A narrow tubular region in the proximal part of the cristae connects the cristae with the limiting region, making the movement of the intermembrane and inner cristae soluble proteins. Cristae are a way to greatly increase the membrane surface to accommodate more respiratory complexes and ATP synthases. In hepatocytes, the cristae membrane may contain 1/3 of the total cell proteins. The inner and the outer membranes are connected by protein complexes found at particular sites in the peripheral double membrane. It seems that cristae are relatively independent of one another and form separate domains. For example, two cristae may show different membrane potentials (charge concentration gradients across the membrane), even in the same mitochondria.
DNA, ribosomes, and many enzymes are located in the mitochondrial matrix, where several metabolic pathways take place. The mitochondrial DNA is found in places referred to as nucleoids, which may contain more than one DNA molecule. Nucleoids are attached to the inner membrane by MitOS complex proteins and are condensed by TFAM proteins. Nucleoids also contain proteins for replication and DNA repair. The DNA contains about 16500 paired bases and around 37 genes that, in humans, code for 13 proteins of the respiratory chain, 2 ribosomal RNAs, and 7 transfer ARNs, which are enough for protein synthesis. Hundreds of copies of the mitochondrial DNA can be found in one cell. Mitochondrial DNA replication is not coupled to the cell cycle, and it can be replicated at any time in the life of a cell. Mutations in one mitochondrial DNA molecule may happen, and therefore there will be DNA molecules with different DNA sequences in the same cell, a situation known as heteroplasmia.
Mitochondria, or parts of the mitochondrial network, are moved through the cytoplasm. They show an extraordinary mobility and are targeted to energy- or calcium-demanding regions of the cell (see below). This is particularly important in neurons, where mitochondria have to be moved to very distant dendrites and terminal axons, from where they can return to the neuronal soma. The movement is not continuous but saltatory, which means that it is stalled from time to time. Long-distance movements are ruled by microtubules, whereas local movements depend more on actin filaments. Of note, both microtubules and actin filaments may also function as anchoring points that stop mitochondrial movement. Within the neuronal axons, the speed of the mitochondrial movement along microtubules is around 0.1 to 1.4 µm/s. This is a fast movement. A slower movement is also observed in growing axons of about 50 µm/h.
Mitochondria communicate with one another by releasing molecules, through membrane contact sites, and by fission and fusion. There are also narrow nanotubules that transiently connect nearby mitochondria. Nanotubules are formed by two membranes and directly connect mitochondrial matrices. They are around 40 to 200 nm in diameter and 1 to 30 µm long. Kinesines protein motors and microtubules are involved in the formation of nanotubules. A defective regulation of calcium leads to stress and the appearing of nanotubules in still mitochondria.
2. Fusion and fission
Mitochondria often divide and fuse with each other, mixing their DNA molecules. It can be said that mitochondria may be found as independent organelles or as a more or less complex mitochondrial network containing many DNA molecules. If two cells are fused, the mitochondrial network becomes homogeneous in 8 hours. Fusion and fission of mitochondria are complex mechanisms because the two mitochondrial membranes are involved, and they must be opened and sealed correctly. The number of individual mitochondria in a cell may change because of this behavior. However, the total mitochondrial volume in a cell seems to be related to the cell volume.
The presumed functions of fusion and fission are to share the synthesized molecules with distant regions of the mitochondrial network, minimize local damages, and share the DNA. There is evidence that mitochondrial fusion is boosted when the cell is under moderate stress, so it has a protective role. On the other hand, it is known that mitochondrial fission increases when there is an acute stress or the cells are close to death. The protection provided by fusion may depend on two mechanisms: fusion inhibits apoptosis and forms a long-distance electrochemical gateway that communicates the interior and the periphery of the cell. In addition, mitochondrial metabolites and proteins are mixed, which buffers local oscillations in molecular concentrations. Anyway, too much fusion may also lead to cell malfunctioning. Under normal physiology, fusion and fission are balanced in the cell.
The division of mitochondria is mediated by DRP proteins (dynamin related proteins; Figure 3). In mammal cells, the endoplasmic reticulum is found in the region where mitochondrial division is going to happen. Before the split and after recruiting DRP proteins, there is an initial constriction of the mitochondria by actin filaments. The nucleoids gathered in these splitting regions. The role of the endoplasmic reticulum appears to be related to correct DNA segregation and lipid transfer between the endoplasmic reticulum and mitochondrial membranes. The lipid exchange seems to be important for synthesizing some lipids like phosphatidylethanolamine and cardiolipin.
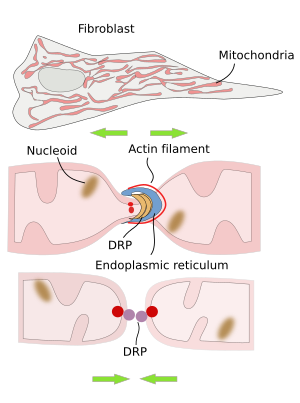
3. Protein import
Mitochondria have a few genes compared to the large variety of protein types they contain. A yeast mitochondria contains around 1000 protein types, whereas it contains around 1500 in humans. Only a few proteins are expressed by mitochondrial DNA, and therefore most proteins have to be imported from the cytosol. This is the result of an intense gene transfer from the mitochondria to the cell nucleus during evolution. It is of note that protein complexes of the respiratory chain are made up of some proteins synthesized in the mitochondria and others in the nucleus. Thus, the nucleus and mitochondria need to coordinate the expression of those genes. Importing proteins is a complicated process since there are several compartments in the mitochondria where proteins can be targeted: external membrane, intermembrane space, inner membrane and mitochondrial matrix. The imported proteins bear amino acid sequences that are like a mailing address, informing the mitochondrial transporters where these proteins have to be delivered.
4. Functions
The primary function of mitochondria is to synthesize ATP, the fuel for most cellular processes. Another function is β-oxidation, which is part of fatty acid catabolism. They also work as compartments for calcium storage, the formation of iron-sulfur groups, and the synthesis of amino acids and heme groups. Mitochondria are involved in apoptosis, cancer, aging, pathologies like Parkinson's disease and diabetes, and the immune responses. On the other hand, comparative studies of the mitochondrial DNA are very useful in anthropology for deciphering genealogies and in other phylogenetic studies because mitochondria are inherited through the maternal lineage and recombination does not occur in mitochondrial DNA during sexual reproduction.
ATP synthesis
Most ATP in eukaryotic cells (in non-photosynthetic eukaryotes) is synthesized in mitochondria. The citric acid metabolic cycle uses acetyl-CoA to produce CO2 and NADH. Electrons from NADH are transferred to the electron transport chain of proteins located in the cristae membrane. Electrons are finally transferred to oxygen to form water. The transport process of electrons through this chain of proteins is able to move protons across the cristae membrane, from the mitochondrial matrix to the cristae inner space. A gradient of protons is generated, which is used by the ATP synthase to produce ATP. ATP synthase is a transmembrane protein located in the cristae membrane. This metabolic process is referred to as oxidative phosphorylation because the oxygen is the last electron acceptor, and it chemically joins inorganic phosphorus and ADP into ATP. In aerobic bacteria, this process is carried out in the cell membrane.
The proteins of the electron transport chain and ATPases are part of the cristae membrane. The ability of the inner membrane to fold many times, forming cristae, is a way to increase the platform where all these proteins work and to keep them close to one another, so the efficiency of the reactions is higher. The many copies of the protein complexes of the electron transport chain and ATPases may account for 80 % of the total weight of the mitochondrial inner membrane.
The set of proteins that carrie out the electron transport is known as the respiratory chain (Figure 4). It is made up of about 40 proteins, 15 of which are for electron transport. Most of these proteins are grouped into three complexes: the NADH dehydrogenase complex, the b-c1 cytochrome complex, and the cytochrome oxidase complex. All have mechanisms to extrude protons from the mitochondrial matrix to the cristae inner space across the inner mitochondrial membrane, a process coupled to the transport of electrons.

The travel of electrons begins when a hydrure ion is released by NADH in the NADH dehydrogenase complex. Two electrons and a proton result from the hydrure ion. The two electrons are gathered by the NADH complex, and they display another two electrons that are transferred to the b-c1 complex by an ubiquinone. Two protons, one in each complex, are extruded to the cristae inner space during this process (Figure 4). The electrons are then accepted by cytochrome C, which passes them to the oxidase complex. In this third complex, another proton is sent to the cristae inner space. Finally, the electrons are transferred to oxygen to obtain water molecules.
The electron transfer from one molecule to the next is a mechanism analogous to batteries, where electrons travel from a place with low affinity to another with higher affinity. This jump releases energy. In mitochondria, the energy is used to move protons against a concentration gradient across the cristae membrane. The electrons in the NADH molecule are retained weakly and therefore jump to the NADH complex. Without the respiratory chain complexes, the energy released by these electrons is lost in heat form. The result of the respiratory chain is a proton gradient, with a proton concentration in the cristae inner space ten times higher than in the mitochondrial matrix. In addition, the negative electric charge of the matrix is higher compared to the inner cristae space because of the change in the proton concentration. Finally, an electrochemical gradient is generated that forces protons to cross the membrane and enter the mitochondrial matrix.
The ATPase forms a hydrophilic passage through the cristae membrane that communicates the cristae inner space and the mictochondrial matrix. Through this passage, the protons are propelled by the electrochemical gradient. The energy of this proton flux is coupled to ATP synthesis. ATPase is evolutionary conserved and can be found in prokaryotes and chloroplasts. It is a huge protein complex made up of several subunits. The ATP synthesis process is very complex and not fully understood yet. However, it is known that for each ATP molecule, the movement of three protons is needed, and that ATPase is able to synthesize 100 ATP molecules per second. An interesting property of ATPase is that it can do the process in the opposite direction, that is, using ATP to pump protons across the membrane.
ATP synthesis is not the only purpose of the proton gradient. Other electrically charged molecules, such as pyruvate, ADP, and inorganic phosphorus, have to be pumped into the mitochondrial matrix, whereas ATP, which is released into the mitochondrial matrix, has to be translocated to the cytosol. Pyruvate and inorganic phosphorus get into the matrix, coupled to the inward flux of protons by symport transport. However, ADP enters the matrix coupled to the ATP exit by antiport transport.
Lipid metabolism
A significant amount of cell lipid synthesis takes place in the mitochondria. They synthesize lysophosphatidic acid, from which triglycerides are synthesized. Phosphatidic acid and phosphatidylglycerol can also be synthesized in mitochondria. Phosphatidylglycerol is needed for the synthesis of cardiolipin and phosphatidylethanolamine.
Cellular stress
Starvation, high temperature, loss of proteostasis, oxidative substances, and many other factors cause cellular stress. Cellular sensors detect the loss of cellular homeostasis and initiate cellular stress responses. When the stress is caused by mitochondria malfunctioning, such as unfolded protein, DNA damages, flaws in the electron transport chain, or mitochondria are affected by other types of stress, the unfolding protein response (UPRMT) is initiated in mitochondria. The UPRMT is a signaling pathway that is linked with nuclear gene expression for recovering the homestasis state. Mitochondria and nucleus are connected through the ATF5 factor. Under physiological functioning, ATF5 is stored in the mitochondria. However, after UPRMT activation, ATF5 is not allowed to enter mitochondria and is then translocated to the nucleus, where it activates the expression of genes that help to recover the mitochondrial homeostasis. Genes to perform glucolysis are also expressed, but the expression of those for the proteins of electron transport chain is decreased. There are other factors involved in the mitochondria-nucleus connection during the stress response.
Mitochondrial stress may be ameliorated by removing defective mitochondria by macroautophagy, a process called mitophagy. The protein importing mechanisms are affected in stressed mitochondria, and proteins to be imported concentrate in the outer mitochondrial membrane, where they are modified and recognized by autophagy. These mitochondria are later degraded by lysosomes. Mitophagy decreases the total mitochondrial volume of the cell. Low oxygen concentration in the cell leads to the activation of the HIF-alfa factor, which in turn induces the expression of NIC and BNIP3, proteins that localize in the outer mitochondrial membrane and are a signal for degradation by autophagy. However, mitophagy may also be a physiological mechanism for cell differentiation. For instance, all mitochondria are removed by mitophagy during the transition from reticulocyte to erythrocyte because some molecules, such as NIX, are gathered on the outer mitochondrial surface and are a signal for organelle degradation. In addition, after fertilization, sperm cell mitochondria that enter the oocyte cytoplasm are selectively degraded by autophagy.
During the normal metabolism of mitochondria, the electron transport chain produces reactive oxygen species (ROS). They are oxidative agents that may lead to oxidative stress if physiological levels are exceeded. However, ROS are not always damaging, and it is now known that they are involved in mitochondrial homeostasis, differentiation, and participate in the mitochodrial stress response.
Cardiolipin and phosphatidylethanolamine are involved in the mitochondrial stress response. Cardiolipin is a sensor of ROS because it is easily oxidized. If oxidized cardiolipin is not degraded, it becomes a messenger of mitochondrial stress because it is exported from the inner to the outer mitochondrial membrane, where pro-apoptotic proteins, such as procaspase 8, Bid and BAX, are recruited. Oxidized cardiolipin also interacts with proteins that initiate macroautophagy.
Apoptosis
Mitochondria are a key organelle that begins the intrinsic pathway of apoptosis (regulated cell death), usually after a non-solved cellular stress. Under intense stress, the BH3-only proteins are activated, which in turn activate and oligomerize the BCL-2 pro-apoptotic proteins BAX and BAK. These two proteins form pores in the mitochondrial outer membrane that let cytochrome C exit the mitochondria. In the cytosol, cytochrome C associates with the proteins apaf-1 and caspase 9 to form the macromolecular complex called the apoptosome. The apoptosome is responsible for the initiation of caspases activity and, therefore, degradation within the cell.
Others
In some species, mitochondria have evolved into organelles with particular functions. For instance, hydrogenosomes are related to hydrogen metabolism, and mitosomes are involved in sulfur metabolism. These organelles do not contain DNA. Recently, mitochondria, together with the endoplasmic reticulum, have been involved in the generation of peroxisomes.
5. Mitochondrial renewing
The mitochondrial population is steadily renewing by removing and forming mitochondria. New mitochondria can only be formed by splitting existent mitochondria and can be removed by macroautophay (specifically known as mitophagy), a cellular mechanism to remove large amounts of cytoplasmic content.
Some proteins are involved in the generation of new mitochondria. PGC-1alfa proteins stimulate the expression of several genes, such as NRF1 and NRF2. These two genes favor the expression of nuclear and mitochondrial genes involved in mitochondrial proliferation.
-
Bibliography ↷
-
Friedman JR, Nunnari J. 2014. Mitochondrial form and fucntions. Nature. 505: 335-343.
Kiefel BR, Gilson PR, Beech PL. 2006. Cell biology of mitochondrial dynamics. International review of cytology. 254: 151-213.
MacAskill AF, Kittler JT. 2010. Control of mitochondrial transport and localization in neurons. Trends in cell biology. 20: 102-112
Palade GE. 1953. An electron microscope study of the mitochondrial structure. Journal of histochemistry and cytochemistry. 4: 188-211. https://doi.org/10.1177/1.4.188.
Pickles S, Vigi P, Youle RJ. 2018. Mitophagy and quality control mechanisms in mitochondrial maintenance. Current biology. 28:R160-R185
Shen K, Pender L, Bar-Ziv R, Zhang H, Wickham K, Willey E, Durieux J, Ahmad O, Dillin A. 2022. Mitochondria as Cellular and Organismal Signaling Hubs. Annual review of cell and developmental biology. 38: 179-218.
-