1. Asymmetry
- Lipids
- Carbohydrates
- Proteins
2. Fusion
3. Repairing
Asymmetry
Cell membranes are made up of two lipid hemilayers. In the plasma membrane, and in the organelle membranes, there is one hemilayer facing the extracellular space, or the lumen of the organelle, respectively, while the other hemilayer usually faces the cytosol. Lipids, carbohydrates and peripheral proteins are present in different types and proportions when both hemilayers are compared. Moreover, transmembrane proteins are placed in the membrane with a precise orientation. This unequal distribution of molecules between both hemilayers is referred to as membrane asymmetry, and is known even before the fluid mosaic model of membrane was proposed in 1972.
Ienerate and maintain membrane asymmetry is essential for the cell. The majority of lipids in the outer hemilayer of the plasma membrane bear choline, such as phosphatidyl choline and sphingomyelin, whereas the inner hemilayer contains more phosphatidyl ethanolamine, phosphatidyl inositol and phosphatidyl serine. The presence of some lipids in a particular hemilayer is important for cell functions. For example, phosphatidyl inositol is mostly located in the inner hemilayer of the plasma membrane and can be split into two molecules by some phospholipases, one of the molecules travels through the cytosol as a second messenger. In addition, the asymmetry determines an unequal electrical charges distribution at both sides of the membrane, which helps to generate the membrane potential. It also allows the association of specific proteins with a particular surface of the membrane due to the distinct environment created by the electrochemical properties of lipid heads, which is different between both sides. The membrane asymmetry also provides physical properties to membranes. For example, in the plasma membrane, it is thought that the formation of vesicles is easier toward the cytosol, i.e., membrane invaginations are favored toward the cytosol.
Disorganization of the membrane asymmetry is sometimes a signal indicating that something is going wrong in the cell. For example, during apoptosis (regulated cell death), the outer hemilayer of the plasma membrane exhibits lipids that are typical of the inner hemilayer. These lipids are recognized by macrophages that finally remove the cell. In fact, there are proteins in the plasma membrane of healthy cells surveying for phosphatidyl serine in the outer hemilayer. When they find one, it is quickly returned to the inner hemilayer. The loss of asymmetry is also important for blood coagulation. Some virus having a membrane envelope show phosphatidyl serine in the outer hemilayer so that they can be incorporated more easily by phagocytosis or macropinocytosis.
AAlthough membrane asymmetry is largely related to the lipid distribution, there is a differential distribution of carbohydrates and proteins between the two hemilayers of cell membranes as well. Carbohydrates are mostly present in the outer hemilayer of the plasma membrane, forming the so-called glycocalyx, and in the luminal hemilayer of endosomes and lysosomes. Carbohydrates work as recognition sites and as a protective layer in the surface of membranes. Proteins also contribute to membrane asymmetry because they are precisely oriented in membranes. Transmembrane proteins have a cytosolic domain and a extracellular domain. This is very important for receptors that need to expose the recognition site to the extracellular space to bind their ligands.
Where and how is asymmetry generated?
Lipids
The asymmetry of lipids (Figure 1) is mainly generated in the Golgi apparatus, but also in other compartments. Curiously, in the endoplasmic reticulum, where most lipids are synthesized, there is a quite similar distribution of lipids between both hemilayers. It is hard for lipids with large polar heads to move from one hemilayer to the other (known as "flip-flop" movement) because of the hydrophobic environment of fatty acid chains. However, lipids with small polar heads, like cholesterol, diacylglycerol, ceramide or protonated fatty acids, swap between hemilayers more frequently. Lipids with large polar heads can save the hydrophobic barrier thanks to specific transporters, or translocases, found in membranes. There are three types of translocases: flippases, floppases and scramblases (Figure 2). These proteins move lipids between both hemilayers, thus modifying the membrane asymmetry. Flippases move lipids to the cytosolic hemilayer, floppases to the non-cytosolic hemilayer (outer hemilayer of the plasma membrane or luminal hemilayer of organelles), and scramblases exchange lipids between both hemilayers. Scramblases are the only one that does not need ATP. The asymmetry is maintained in the cell membranes because "flip-flop" movements are rare. For instance, most sphingolipids are not exchanged between hemilayers by these translocases, and they remain in the hemilayer where they were synthesized: the luminal hemilayer of the Golgi apparatus, which becomes later the outer hemilayer of the plasma membrane. More than 80 % of the plasma membrane sphingolipids are located in the outer hemilayer.
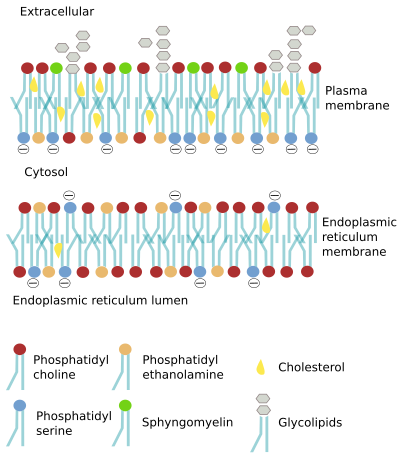
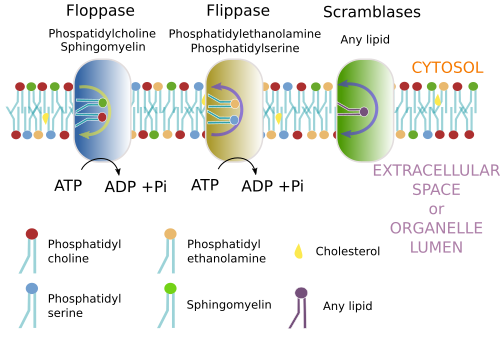
Translocases are selectively distributed through the membranes of cell compartments, providing particular local asymmetry. Moreover, the distribution and activity of members of the same family are also regulated. As mentioned previously, apoptosis changes the asymmetry of plasma membrane, initiating phagocytosis. This disorganization of membrane asymmetry is produced by the activation of a scramblase located in the plasma membrane. However, the scramblases located in the endoplasmic reticulum are always active and are responsible for the symmetry found in their membranes. In the erythrocyte membrane, scramblases are activated after massive calcium entry and favor to blood coagulation.
Carbohydrates
Carbohydrates are found in the outer hemilayer of the plasma membrane and in the luminal surface of endosomal/lysosomal system. Carbohydrates are synthesized in the membranes of the endoplasmic reticulum and Golgi apparatus. In the endoplasmic reticulum membrane, the initial steps of carbohydrates synthesis begin in the cytosolic surface, but it continues in the luminal surface. In the Golgi apparatus, all steps of carbohydrate synthesis steps are done in the luminal surface.
Proteins
Transmembrane proteins are arranged and oriented in the membrane during the synthesis process in the endoplasmic reticulum. Proteins associated to the cytosolic hemilayer are synthesized in the cytosol by free ribosomes.
2. Fission and fusion
A very useful feature of membranes is their ability to split and get fused again. It provides an extraordinary plasticity for remodeling membrane bound compartments, which means grow, division, and fusion of these compartments. They are also able to release and recruit vesicles. These features are indispensable for vesicular trafficking and for cell division, particularly during cytokinesis, when plasma membrane increases in size, breaks, and get sealed again to form the two new cells. Membrane fission and fusion are largely ruled by proteins, such as SNARE (soluble N-ethylmaleimide-sensitive factor attachment receptor). The physicochemical properties of lipids are also involved in these processes.
Repairing
A number of natural processes and experimental handling may lead to membrane breakages. For example, some tissues are under natural mechanical forces that compromise the plasma membrane integrity, as it usually happens in muscular tissue. Cardiomyocytes undergo frequent minor breakages of their plasma membranes that are repaired quickly. In the laboratory, cell cloning protocols need to introduce glass micropipette tips into the oocytes through the plasma membrane, electroporating of membranes is needed to introduce DNA or vectors in the cells, handling of cells in cultures produces mechanical loads that break cell membranes. Holes in the plasma membrane are lethal for the cell if they are not sealed in a few seconds. Cells have repairing mechanisms to prevent the loss of the internal content, and thus maintain an internal environment different from the external one. In some tissues where cells do not proliferate, like the nervous tissue, replacing the damaged cells is not an option. Therefore, they must have effective membrane repairing mechanisms. mechanisms.
There are two mechanisms for repairing cell membrane breakages. When the gaps are small (smaller than 0.2 µm) (Figure 3), the physicochemical properties of lipids are able to repair the damage. At the edge of the membrane gap, lipids change to an unstable arrangement that pushes the edges against each other, eventually closing the hole. How quick is this sealing depends on the membrane tension, which in turn depends on the anchoring points between the plasma membrane and both cytoskeleton and extracellular matrix. When a gap is made in the plasma membrane, calcium enters into the cytosol propelled by concentration gradient. The increase of calcium in the cytosol partially disorganizes the cytoskeleton around the damaged area, reducing the number of contacts between cytoskeleton and plasma membrane. The membrane tension is loosened, and, it is therefore easier and faster to seal the gap. ESCRT proteins, which are involved in the formation of intraluminal vesicles in the multivesicular bodies, are also involved in the repairing of small membrane breakages (less than 100 nm). In in vitro membrane models, it has been frequently observed very small holes (a few nm) as a consequence of the normal lipid movement by fluidity, which are quickly sealed by the lipids properties. This is not regarded as membrane damage and repair but a normal behavior of the membrane.

When damages are larger (more than 0.2 - 0.5 µm), the edges of the gap are too far from each other and no auto-sealing is possible. In this scenery, a cellular response is initiated (Figure 4). The repairing process must last no more than a few seconds, because cell dies after that time period. A large gap allows the entering of a huge amount of calcium that changes the cellular behavior. However, if the calcium concentration is not restored by sealing the breakage, there is cell content loss and apoptosis is activated. It has been shown that sea urchin oocytes are not capable of repairing their membranes in calcium free environments, and therefore die. It is curious that sodium, potassium or chloride ions do not participate in this repairing process. The initial increase of calcium triggers the repairing mechanisms, which include the fusion of membrane bound compartments located in the cell cortex with the plasma membrane, working like a patch. The compartments involved are endosomes, lysosomes, vesicles and other organelles. Lysosomes are thought to be important in this process. Endocytosis and endoplasmic reticulum disorganization would help to form more compartments used for the sealing. In addition, some proteins get oxidized and make bridges between vesicles near the gap, keeping them close for patching. Calcium also activates enzymes, like proteases, that help in the sealing process by removing the cytoskeleton from the affected area and making easy the movement of organelles toward the area. Actin filaments get organized like a ring around the borders of the gap. This ring gets smaller in diameter dragging the edges of the gap and new membrane to the center of the breakage. From an evolutionary point of view, it has been suggested that this repairing mechanism was later modified during evolution and used for fusion and fission of inner membranes and for vesicular trafficking.
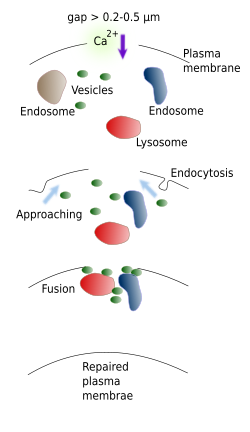
The mechanism of fusion of the inner compartments between each other to form a large compartment before the fusion with the plasma membrane to repair the gap, suggested by McNeil, has not experimental support. This supposed inner large compartment has not been observed at electron microscopy and membrane action potential measurements fit best with a progressive fusion of many small inner compartments with the plasma membrane. Moreover, the sealing is faster in those cells with many membrane-bound organelles.
CCells and tissues develop molecular mechanisms to get adapted to repetitive mechanical stretching: the extracellular matrix changes its molecular content, cells increase the number of adhesion molecules in plasma membranes, synthesize more intermediate filaments for the cytoskeleton, and increase the number and size of membrane-bound organelles. Cellular responses to mechanical stress can be studied in cell cultures. It has been observed that stretching of about 10 to 15 % increases the membrane surface by fusion of internal organelles. This usually happens in the urinary bladder epithelium, which frequently undergoes large stretching. If cells in cultures are stretched twice, a faster repairing process can be observed the second time when compared with the first one. Furthermore, the amount of vesicles released by Golgi apparatus is larger than usual, so that the cell may respond more efficiently when membranous compartments need to be fused between each other. The cell attachment to the extracellular matrix is also strengthened. The proteins of the cytoskeleton and extracellular matrix increase in number to withstand repetitive mechanical tensions.
MMembrane repairing and cellular regeneration are not the same processes. Membrane repairing mechanisms are started after a membrane damage. Cell regeneration begins when a large part of the cell is loss, so that the damaged cell must synthesize and assemble molecules to restore the normal state.
-
Bibliography ↷
-
Bibliography
Bittner GD, Spaeth CS, Poon AD, Burgess ZS, McGill CH. 2016. Repair of traumatic plasmalemmal damage to neurons and other eukaryotic cells. Neuronal regeneration research 11:1033-1042.
Daleke DL. 2007. Phospholipid flippases. The journal of biological chemistry. 282:821-825.
McNeil PL, Steinhardt RA. 2003. Plasma membrane disruption: repair, prevention, adaptation. Annual review in cell and development biology. 19:697-731.
Nicolson GL. 2014. The Fluid—Mosaic Model of Membrane Structure: Still relevant to understanding the structure, function and dynamics of biological membranes after more than 40years. Biochimica et Biophysica Acta (BBA)-Biomembranes. 1838(6): 1451-1466.
Quazi F, S. Molday RS. 2011. Lipid transport by mammalian ABC proteins. Essays in biochemistry. 50, 265–290.
Tang SKY, Marshall WF. 2017. Self-repairing cells: How single cells heal membrane ruptures and restore lost structures. Science. 356, 1022-1025.
-